

The peak with the highest binding energy at about 289.4 eV can be attributed to O=C–N bonds 28 or other carboxyl containing precursor residues. Three peaks are sufficient to fit these spectra, with binding energies centered around 285.2–285.5, 287–287.3, and 289.2–289.5 eV. 3, the carbon C 1s spectra of SnO 2 layers deposited at different temperatures are shown.
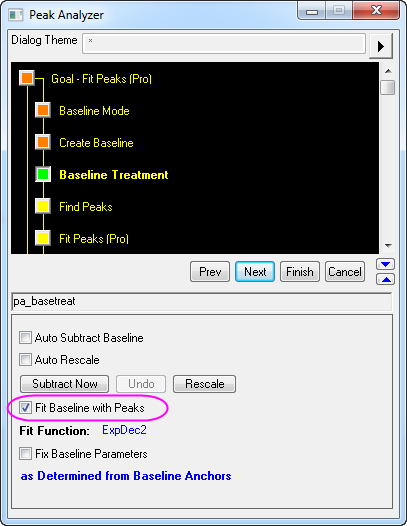
We proceed by discussing the carbon C 1s and nitrogen N 1s core levels. However, the trend with temperature change is similar in both the optimized and nonoptimized sets of samples. Note that the relative area of other species at low temperatures for the optimized cycle is almost comparable with the area for high temperatures using the nonoptimized cycle. This decrease is more prominent for the not optimized cycle parameters, where the relative area of other species at the lowest temperature was above 20%. For both the optimized and the nonoptimized sets of deposition parameters, with the increase of the temperature, there is a decrease of other species, which include nitrogen, carbon residuals, and -OH groups. 7) as “oxygen,” and the sum of the area of the C 1s, N 1s, and O 1s secondary peak as “other species” (see discussion below for more details). For this plot, we label the total peak area of Sn 3d 3/2 core level spectrum as “tin,” the area of the main peak in the O 1s spectra (see discussion related to Fig. 2 shows the relative peak areas of core levels that are present in the SnO 2 for both processes (“not optimized” and “optimized”). 39 Before discussing the core level spectra in more detail below, we present an overview of the findings: Fig. Details regarding the peak fitting of all XPS data from SnO 2 layers with different deposition conditions are presented in the supplementary material. The XPS data analyzed for these SnO 2 layers include tin 3d 3/2, oxygen 1s, carbon 1s, and nitrogen 1s signals. For the optimized cycle, the SnO 2 layers show similar conductivities and a comparable trend versus deposition temperature as reported for thermal ALD using the same precursor. Thus, for the sample of the not optimized process deposited at 120 ☌, no resistivity could be measured. At low deposition temperatures, this resistivity even increased to ∼10 5 Ω cm, i.e., the detection limit of the used measurement setup. The resistivity of samples deposited using the optimized cycle saturates at about 10 mΩ cm at a deposition temperature of 165 ☌, while the resistivity of samples deposited with the not optimized process is more than 3 orders of magnitude higher. 1(a) both trends show similar behavior over temperature, with a drastic decrease of resistivity by about 4–5 orders of magnitude when changing the deposition temperature from 120 to 200 ☌. For the electrical properties, as shown in Fig. 1 shows a variation in the electrical and optical properties of the deposited SnO 2 layers as a function of the deposition temperature.
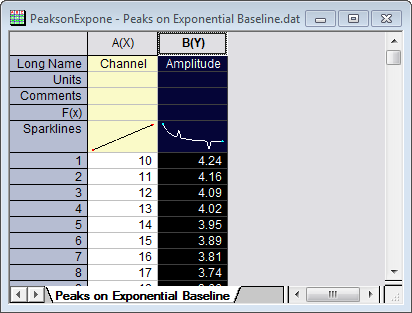

The parameters for this dataset are deposition temperature of 120 ☌, RPS power 300 W, and the oxygen duration time of 2–8 s.įor these two parameter sets, Fig. 39 Additionally, for the investigation of byproducts influence with XPS, the dataset for the plasma duration optimization was used. 24 More detailed information on cycle optimization is provided in the supplementary material. For longer durations or higher plasma power density, there is a possibility of plasma-induced defects inside the material or at the surface 23 and the increase of energetic radical concentration that could remove reactive sites from the substrate. After the optimization, the optimum oxygen step duration was established at 6 s, at an RPS power of 300 W. The initial (not optimized) cycle had the oxygen step duration of 2 s, with RPS power of 150 W. Moreover, for further improvement of the plasma's reactivity, an additional flow of 10 sccm argon was introduced into the chamber during the oxidation step. Therefore, the parameters of the oxidation step (pulse time, plasma power) were optimized with regard to conductivity of the SnO 2 layer. If the reaction kinetic is slow and plasma species are distributed isotropically, 22,23 it is possible to obtain better quality layers before the saturation point. However, for PEALD, the saturation point for the plasma step cannot be adjusted in the same way as for thermal ALD mostly due to plasma kinetics. TDMASn and N 2 pulses were optimized to yield saturation of the film growth per cycle (GPC).
